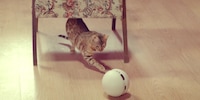
News + Trends
Introducing the cat tormenter and other quirky gadgets from the future
by Aurel Stevens
«This battery makes your smartphone last a whole week»; «It charges within 15 minutes». Either of these statements sound familiar? It’s as though not a month goes by when we don’t hear about a revolutionary battery that’s going to be launching really soon. I think it’s time for a reality check.
At the beginning of 2016 Philipp Rüegg and I were in charge of the modest editorials for digitec and Galaxus. One of my first articles covered the Mobile World Congress (MWC) in Barcelona. Fast-forward to today and there are 15 of us on the team. Dominik Bärlocher has taken over the world of smartphones, which, to be fair, he does much better than me. But that’s beside the point.
Back in the old editorial days, the company MyFC showcased the JAQ powerbank. This was a replaceable fuel cell that allowed you to charge your smartphone without a power outlet thanks to handy cartridges. Pretty cool, huh?
It’s now two years later. What happened to JAQ? Their website is littered with new press releases. But, sadly, you still can’t catch a glimpse of the product in shops. It’s the same story for the NEC notebook and its fuel cell that was in the prototype stages as far back as 15 years ago.
Does that make fuel cell a dead technology? Of course not. The car manufacturer Toyota, for one, has high hopes for it. While the company might not have had resounding success with fuel cells, they have reason not to throw in the towel just yet. Fuel cells remain a portable energy storage that has the potential to take the market by storm.
Talking of the automotive industry, Tesla & Co are driving the already huge demand for improved energy storage systems and batteries in particular. Just as they are for photovoltaics, a technology that is becoming increasingly widespread and attractive in terms of price. The world is waiting with bated breath for the next generation of batteries.
To prevent unnecessary disappointment, let's start with some theoretical background. With this in mind, you'll know what to expect when you read the news reports («innovative new battery is here!») and the small print («coming to the market in a few years»). It’s often the case that interim stages in research and lauded materials are hyped as «the next big thing».
Is it just me, or can you feel an example coming on?
That’s the headline and here is the small print right at the end of the article – where else:
It is as yet unclear when graphene batteries are going to be produced on a large scale and turned into an end product.
As a general rule, the press like to paint an overly optimistic picture of research findings because it results in a juicy headline. But the reality is, most concepts end up getting binned. Laboratories obviously don’t want to make a big song and dance about any failings. But even that type of research isn’t in vain. After all, it’s just as important to know what doesn’t work.
While we’re on the subject on research, it’s worth mentioning that battery development requires a great deal of perseverance. It takes ten years or more to take a concept or product from the lab to the shelves of an online shop.
Building an efficient assembly line alone can take several years. End product manufacturers have to adapt their goods, chargers and processes. Authorities also insist on extensive safety checks, and so on. At the end of February 2018, the world’s major car part supplier, Bosch, decided against producing its own cells because it was too expensive.
Any new batteries obviously have to be an improvement on what’s currently available. Otherwise there would be no incentive for the expensive affair that is inventing the next innovative battery. These also have to meet a variety of requirements for each intended purpose as best as possible:
Wow. We really do ask a lot of batteries.
Depending on their intended use, batteries might even be expected to have other characteristics. At the moment, car batteries use lead and sulphuric acid because it’s cheap and it doesn’t matter that they're rather heavy. You also get home batteries that are supposed to store solar energy. These kinds of batteries are kept in the basement, so it doesn’t matter if they take up a lot of space in exchange for being cheaper. However, when it comes to batteries for mobile devices, these have to be compact and as light as possible.
Here’s the tricky bit: when you optimise a battery in favour of one characteristic, it usually means compromising another. That’s illustrated well in the Samsung example above. The graphite battery can be charged lightening-fast and it has high capacity thanks to the special material. However, this is at the expense of the size. The volumetric density of graphite balls is much higher than conventional graphite. (Incidentally, that article was authored by all of the Samsung researchers – the very same ones COMPUTERBILD wrote about in an article detailing the lessons they’d learnt.)
To avoid getting tricked by the headlines, you need to be able to recognise the things that are going to make progress possible. To do that you need to understand how and why a battery works. So you see, we still need to cover a bit more theory. It’s time for a detour into electrochemistry. Feeling nervous yet? Don’t worry. It might be on the complicated side but I’ll stick to the essentials.
The operating function of any battery is easy to explain, so let’s start off there. When you connect a load, such as a light bulb, to a battery, the stored chemical energy is converted into electrical energy. Unlike single-use batteries, rechargeable batteries can also work in the other direction, in other words transforming electrical energy to chemical energy.
Various concepts and materials can be involved, but it’s always the same parts that are used, namely two electrodes, one electrolyte and a separator. The chemical reaction is caused by attaching the load. More specifically, the load completes the circuit and allows electrons to flow.
One electrode is called the anode, while the other is the cathode. When the battery discharges, the anode is the minus pole and the cathode is the plus pole. However, the poles switch during charging. That’s why it’s clearer to stick to the terms anode and cathode.
The anode requires a chemical reaction that emits electrons. This results in positively charged atoms or ions. These ions want to be negatively charged again so they travel through the electrolyte. The cathode is happy to accept electrons and transform into another molecule. The ions then pass through the separator and are reunited again.
The change in charge after a reaction in a molecule is called potential, which is measured in volts. You can see the potential that molecules have by consulting the electropotential series. When you look at the table, you’ll understand why lithium is so interesting.
There are two compatible reaction pairs in the battery: electrons flow from the anode to the cathode and the reactions on both sides have to interact with the same ions. The anode emits electrons and leaves behind ions. This chemical process is called oxidation. The cathode accepts electrons and binds ions in a process known as reduction.
The technical term for these processes together is a redox reaction. This means two pairs of chemical reactions that interact as an overall system, or redox pairs.
Chemical reactions take place in the cell as long as there are molecules on both electrodes that still want to emit or accept electrons. As soon as one of the electrodes no longer has molecules to emit, accept and bind electrons and ions, the process grinds to a halt. In other words, the battery is empty. What’s special in this case is the battery can conduct the reaction backwards, by feeding electrons.
If electricity was like water, the potential would be the gradient between both poles. One of them is very high while the other is very low. That’s why the electrons want to «flow down» to the other pole. By contrast, the current depends on how many electrons are actually moving. Current is measured in amps.
Here’s another water analogy: imagine a little stream and a huge river. What sets those two apart is the different amounts of pressure, how steep the gradient is (volts) and how much water is flowing (amps). The product of volts × amps is the power in watts. Power × unit of time (mAh, Wh) equals energy.
This correlation is important. In your mobile phone or notebook, for instance, the capacity is usually given in milliamp-hours (mAh). Using the formula above, you know that mAh doesn’t mean a lot if you don’t know the cell voltage.
Example: you have a nickel-cadmium battery with 1000 mAh and a lithium-ion battery with 500 mAh. Which has a greater capacity? The NiCd battery has a nominal potential of 1.2 volts, while the lithium-ion battery has 3.6 volts. In line with the formula above, watt-hours (Wh) = volts × mAh. You then divide the whole thing by 1000, as we’re working with milliamp-hours. The results are as follows: the NiCd battery saved 1.2 watt-hours of energy, while the Li-ion battery had 1.8 watt-hours.
The potential depends on how full the battery is. When it’s fully charged, the electric potential is much higher than when the battery is almost empty. In a car battery, the potential could range from 1.75 to 2.4 volts depending on how well it was charged.
The desired electric potential has an impact on the structure of the battery. If you’re a driver, you’ll know that a car battery has 12 volts rather than 2. That’s why that kind of battery features six cells connected in series. While the official voltage figure of a car battery is 12, the exact value can range from 10.5 V to 14.4 V. Cell voltage is just one of a number of ways to determine the state of charge.
But connecting in series isn’t the only option available. You can also connect cells in parallel. This increases the capacity rather than the potential. The image above is a good example of that. Look inside each cell and you’ll notice lead and lead oxide plates sandwiched on top of each other.
You can’t charge and discharge a battery interminably – as you can see from the photo of the very old lead battery. Over time, increasingly large PbSO₄ crystals took hold of the once spotless electrodes. This greatly reduced the size of the active electrochemical surface, meaning capacity dropped.
Something similar happens to lithium-ion batteries that damages them in the long run. Over time, charging causes a film to form on the anode that covers the graphite surface. This prevents the graphite interacting with the electrolytes and ions, meaning capacity drops.
Meanwhile, the cathode on Li-ion batteries is subject to an effect known as electrolyte oxidation. That tends to occur when there’s a high cell voltage – in other words, when the cell is fully charged. Lithium-ion batteries are considered to be depleted when they only have 80 per cent of their former capacity. Why then? Because that’s when they start to age a lot faster.
In summary: a lot of chemical reactions aren’t completed. If the battery is fully charged or completely empty, the negative effects can be intensified in a lot of batteries. Moreover, very high or low temperatures and charging or discharging too fast can accelerate processes you really don’t want in the battery. The effectiveness of the chemical reaction is called Faraday efficiency.
In the lab, they try to minimise this kind of collateral damage. To do that, they use new materials or additives that are attached to the electrolytes. That’s also why the anode on a lot of batteries is covered in graphite. It’s a way of ensuring lithium isn’t directly exposed to electrolytes so that any damages are slower to appear.
Battery research is always about:
Now that you’ve got the basics covered, we can get down to the nitty-gritty. What everyone wants to know is which battery technologies are being researched and what makes them so promising.
The chart is very much simplified. In reality, energy density can’t be depicted as a rectangle. It’s better to imagine them as points on a model. For instance, there isn’t just one NiMH battery and not only one type of lithium-ion battery – rather, there are different varieties.
Each model is developed through research so that modern models fall above or to the right in the chart. Ideally, they’d be as high in the upper right corner as possible. You’re more likely to find emerging technologies, such as lithium-sulphur, in the bottom left corner, while the top right corner is the preserve of the projected maximum-impact batteries. I chose to focus on the estimated values in practice rather than the theoretical maximum.
The chart also gives you a clear picture of the history of battery development:
If you compared the energy density of batteries with that of petrol or diesel, the chart would look very different. All of the batteries would be pushed down and towards the left. After all, it’s hard to trump petrol with its 13,000 Wh per litre. But if you zoomed out, everything we talked about would just be a pixel on the bottom left of the chart. Compared with nuclear fission, everything else has energy density about a million times less.
Lithium-ion accumulator
The lithium-ion accumulator is the main type of energy storage, and it has distinct advantages over its precursors. In this type of battery, the memory effect is hardly visible. In other words, they no longer have to be completely charged and discharged. In day-to-day life, that’s a priceless advantage. The batteries are also smaller, more sustainable and cheaper. They’re less likely to self-drain than NiMH batteries, for example, which see a loss of 20 per cent each month.
However, there isn’t just one type of lithium-ion battery. Instead, they come in lots of different guises. What unites them is good energy density and low self-drain. Modern versions can also withstand a good few hundred charge cycles. In terms of use, they’re more than safe enough as long you don’t crush them, as Samsung did in the Note 7. In that instance, the graphite on the cathode got stretched when lithium ions were accepted. When there wasn’t space for them, the battery broke and the highly reactive lithium came into contact with the rest of the battery.
While lithium-ion batteries have a number of benefits, they do also have a lot of drawbacks. They don’t withstand heat well and in high temperatures they break easily. Meanwhile, when it’s below 0° Celsius, they can’t be charged. Deep discharge completely destroys them. That’s why the electronics inside these batteries are uncluttered and they even feature a temperature sensor.
The electronics help the battery to operate within safe parameters and not get damaged. However, they’re also partly responsible for Li-ion batteries losing around three to five per cent of their charge each month, even when they’re not used.
Can I just say here once and for all: please don’t take any batteries apart (neither single-use nor the rechargeable kind). It really can be dangerous! To satisfy your curiosity, here’s what a (replaceable) notebook battery looks like:
Cast your mind back to the battery qualities mentioned at the start of this article. Those characteristics are exactly the reason why lithium-ion batteries come in different forms. Some focus on maximum safety, while others put the emphasis on high cycle-stability.
It’s more than likely your smartphone contains technology using cobalt, or more precisely LiCoO₂ (LCO). It was the first developed model, and its power has been more than doubled since 1991. To set that in context, here are some other common versions:
Lithium polymer batteries
You might recognise lithium polymer batteries, or LiPos for short, from remote control cars. Technically, they should be called lithium-ion polymer batteries, because in electrochemical terms, they’re not much different to their relatives mentioned above. The difference lies in the electrolyte. In traditional Li-ion batteries, this is a liquid, while in polymer batteries it is a solid.
Polymers are fibrous macromolecules that are strung together. They can occur naturally, for example in your hair. But they can also be artificial. The polymer in LiPos is a gelatinous, wobbly material that is a good conductor and can accept a lot of ions.
Meanwhile, solid electrolytes have practical advantages. Thanks to their inherent stability, LiPos can be made in a wide variety of shapes and sizes. Their casing doesn’t need to be as sturdy; a thin, light foil is enough.
Perhaps you’re already aware that RC batteries are particularly delicate. If they get damaged or overloaded, they can blow up and catch alight. That’s why model builders charge and store their lithium polymer batteries in fire-resistant bags to stop their whole flat going up in flames.
But that’s only because energy density was put before safety. To save on weight, safety electronics were also done away with and moved to the charging unit. In theory, lithium polymer batteries can be built just as safely as their lithium-ion counterparts. Just to prove it, lurking beneath the Macbook Pro I’m typing on, there’s a six-cell LiPo.
Lithium-metal batteries
Lithium-metal is the promising successor to lithium-ion technology. It’s already common, just not in this rechargeable form. An example is lithium button cell batteries. Lithium-metal features twice as much energy density as Li-ion batteries with low self-drain.
The approach is based on the idea that lithium without the graphite layer would directly expose the electrolytes. As a result, the chemically active surface area is very large. This translates into high capacity and fast charging. To make the lithium-metal battery more compact, manufacturers can omit the graphite.
However, rechargeable lithium-metal batteries are still not ready to be used in practice. This is because the metal that is directly exposed forms so-called dendrites in every cycle. These are deposits that grow into the electrolytes like a stalagmite and can even pierce through into the separator. As soon as that happens, the battery short-circuits. We’ll know there has been a breakthrough in research when dendrite formation is prevented or slowed.
Solid-state batteries
You hear about solid-state batteries more and more these days. When it comes down to it, they’re just a component that lets us use lithium-metal batteries. The idea is that the electrolyte shouldn’t be a liquid. Instead it’s made of a porous or a crystalline material. Having a solid electrolyte solves the problem of dendrite formation.
It can also be thinner than the combination of liquid electrolyte – separator – liquid electrolyte. That facilitates much more flexible designs, such as thin foil. There’s supposed to be a tendency for solid-state batteries to last longer. On top of that, they’re safer because the electrolyte can’t escape.
You probably know the score by now. There’s usually always a quality or characteristic pushed to the fore at the expense of another. Which one is it this time? In terms of drawbacks to solid-state batteries, lower power is certainly on the list. Present solid electrolytes don’t conduct ions as well as their liquid equivalents. That means the battery takes a bit longer to charge, and the electricity it delivers while discharging isn’t as high.
Moreover, current designs can’t handle low temperatures very well. And prototypes only last a few hundred cycles. To top it all off, recent solid electrolytes are incredibly expensive. A solid-state car battery would set you back tens of thousands of Swiss francs.
Lithium-sulphur batteries
Lithium-sulphur batteries are the potential market successor – they’re bursting with potential. Researchers have been tinkering about with them since the 1960s. They have almost three times the energy density of Li-ion batteries. What’s more, lithium and sulphur are light elements. The chemical reaction also takes place in low temperatures; these batteries can even charge at -60° Celsius. They have the advantage of sulphur being ecologically sound and in plentiful supply.
As with lithium-metal batteries, the lithium-sulphur counterparts don’t use graphite on the anode. This frees up weight and space. Moving on to the cathode, the metal oxide there is replaced with cheap and light sulphur. The chemical reaction is still efficient, as two lithium-ions can attach per molecule.
In the lab, these batteries attained very high values for energy density and power. However, optimised prototypes do break quickly. They only managed a few dozen cycles. In the last few years, researchers pushed that figure up to over 4000 cycles, but this was at the expense of energy density and power.
Lithium-air batteries
Lithium-air batteries have been the focus of research since the 70s. In fact, you could say they’re the holy grail of battery research. There are two reasons for that.
Firstly, they let you save a bunch of space because one of the reaction components (O₂) doesn’t have to be built into the battery. Instead, it’s absorbed through the environment. Secondly, lithium-air batteries have an unbelievably high theoretical energy density at 13 kWh per kg, which is the same as petrol. In practice, it’s expected to reach around 2,800 Wh/kg. Given the relative inefficiency of combustion engines at 30 per cent, a lithium-air battery would be revolutionary in an electric car with an efficiency of 90 per cent.
Realistically speaking, it will probably be a long time before lithium-air batteries are available. A number of researchers even doubt whether they will reach the mass market given the battery’s many problems.
Sodium-ion batteries
In sodium-ion batteries, sodium replaces lithium. Energy density is pretty poor in these models, coming in as it does around 90 Wh/kg. As a result, these kinds of batteries would need to be very big and heavy. However, sodium-ion batteries could still be of interest for uses where space and weight are irrelevant. Sodium is almost indefinitely available, meaning it’s also very cost-effective. In addition, it is safer than lithium. An advantage of sodium-ion is that the chemical reaction is immune to deep discharge.
Ongoing development with graphene
Up until now, graphite has been used on electrodes in Li-ion batteries. Graphite is a form of pure carbon, just like diamonds. Both have a different atomic structure. Meanwhile, graphene is also a pure carbon. The difference is it is structured two-dimensionally as a hexagonal, honeycomb design.
Graphene is awe-inspiring material that has surprising qualities. It is almost 125 times stronger than steel. It’s harder than diamonds and it can be rolled. Graphene is also transparent, impermeable to liquid and a very good conductor.
It’s the latter that makes this material so promising for use in batteries. If the anode was covered in a layer of graphene, it would also last much longer thanks to graphene’s stability. Similarly, if graphene were used as cathode material, the larger surface area would shorten charging times drastically and significantly increase capacity.
In theory, adding graphene to batteries would improve them a great deal. I stress the phrase «in theory».. The hype surrounding graphene is directly proportional to the qualities of this much-lauded material. The problem is that it’s difficult to manufacture graphene in pure form and in large quantities. It will probably be another ten or so years before graphene appears in batteries.
Fuel cells
Fuel cells come in various forms. They can use alcohol compounds, glucose solutions and compromised hydrogen. But what they all have in common is that they convert chemical energy directly into electrical energy with the help of an oxidising agent, unlike the process in combustion engines. In this sense, the name «fuel» cell is slightly misleading.
The most common version is the hydrogen-oxygen fuel cell. It has the advantage of being able to extract one of the reaction components, the oxidising agent, from the environment. I’m obviously referring to oxygen. The product of the chemical reaction is H₂O, and the «waste gas» from the exhaust is water. That sounds crazily clean. At a local level, fuel cells are heralded as clean energy and could thereby completely solve the exhaust fume problem in cities.
Globally speaking, though, fuel cells aren’t an ecological miracle as the whole process, from manufacture of oxyhydrogen gas or methanol to the conversion into electrical energy, is rather inefficient. The efficiency of the whole chain is about 60 per cent. In areas where renewable energy is readily available, this drawback doesn’t carry as much weight.
Fuel cells are indeed used commercially, and the miniaturisation is nearer to reality. The major stumbling block, however, is the infrastructure that’s required. This is aggravated by the fact that the more promising new battery technology is, the less interesting inefficient fuel cells become.
Oops. The article has become a bit longer than I originally intended. But at least you now know how a battery works and in what order you can expect improvements. It’s certainly an industry to watch. And some of the approaches I mentioned are almost within reach. Who knows? Maybe a bold start-up will forge their own path.
In terms of predicting the future, the last point in particular should help you assess the state of the technology. If you’re still charged up and wanting to find out more, I’d recommend taking a look at the batteryuniversity.com website.
I'm the master tamer at the flea circus that is the editorial team, a nine-to-five writer and 24/7 dad. Technology, computers and hi-fi make me tick. On top of that, I’m a rain-or-shine cyclist and generally in a good mood.